Chapter 3 Glycolysis
Figure 3.1: Carbon Reductions
In most living organisms, biosynthetic reactions are driven from the oxidation of organic substrates. Oxygen is a strong electron acceptor and has a high tendency to attract electrons: \[\begin{equation} C_6H_{12}O_6 + 6O_2 \to 6CO_2 + 6H_2O + \text{Energy} \end{equation}\]
3.1 What is “Energy?”
Energy is simply the capacity to cause change. Potential energy is energy that an object has because of its position in time and space (e.g., electrons have potential energy because of their distances from the nucleus).
Changes in potential energy occur in steps:
Figure 3.2: Potential Energy Demonstrations
However, in the context of BS2003, glucose and other molecules are broken down in a series of steps. Electrons from the oxidation of organic compounds are usually transferred to the coenzyme NAD+.
Figure 3.3: NAD+ in Action
3.1.1 Overview of carbohydrate metabolism
Figure 3.4: Structures of Different Sugar Polymers
Starch, lactose, and sucrose are the major carbohydrates in the average human’s diet. Amylose and amylopectin are polysaccharides with hundreds, if not millions of glucose residues linked via \(\alpha\)(1,4) and / or \(\alpha\)(1,6)-glycosidic bonds.
Lactose is a disaccharide that is linked via a \(\beta\)-(1,4)-glycosidic bond and is comprised of glucose and galactose.
Sucrose is a disaccharide that is made up of glucose and fructose monomers; it is linked through an \(\alpha\)-(1,2)-glycosidic bond. Digestion converts all of these dietary carbohydrates into their respective monosaccharides via hydrolyzing glycosidic bonds.
Glucose is the universal fuel for human cells and the source of carbon for the synthesis of most other compounds. The hormones insulin and glucagon are released from the \(\alpha\) and \(\beta\) cells of the pancreas regulate the concentration of sugar in the body.
Other dietary sugars are convered into glucose or at least intermediates of glucose metabolism. These monosaccharides are absorbed from the villi in the small intestine and travel to the tissues (where they are then metabolized):
Figure 3.5: Sugar Processing in Humans
3.1.2 What happens to glucose after it enters the cell?
Glucose is thereafter phosphorylated by a hexokinase to form glucose-6-phosphate - a molecule capable of entering many pathways:
Figure 3.6: Possible Pathways of Glucose-6-Phosphate
However, because glucose-6-phosphate is ionized (i.e., negatively charged) at physiological pH, the plasma membrane is also impermeable to glucose-6-phosphate (and so glucose-6-phosphate is unable to leave or enter cells from the bloodstream).
Fructose and galactose are converted into intermediates of sugar production:
Figure 3.7: Fates of Fructose and Galactose
3.2 Glycolysis (and a little about the TCA cycle)
3.2.1 Overview of glycolysis and the TCA cycle
Glycolysis begins with the phosphorylation of glucose by hexokinase to glucose-6-phosphate. Subsequently, one glucose-6-phosphate molecule is oxidized into two pyruvate molecules and generates two nicotinamide adenine dinucleotide (i.e., NADH) molecules in the process.
A net total of two ATP molecules occurs from the transfer of high-energy phosphate from glycolysis intermediates.
Figure 3.8: Glycolysis and the Krebs Cycle
Glycolysis happens in the cytosol and generates NADH. However, because NADH cannot cross the inner mitochondrial membranes, electrons from cytosolic NADH are transferred to the electron transport chain via the malate-aspartate shuttle or the glycerol-3-phosphate shuttle (see chapter 5).
Pyruvate from glycolysis becomes completely oxidized to CO2 by pyruvate dehydrogenase and the TCA cycle in mitochondria. The aerobic oxidation of glucose to CO2 generates about 30 to 32 moles of ATP per mole of glucose!
3.2.2 Energy investment phase
Figure 3.9: Energy Investment Phase
The phase itself begins with the transfer of a phosphate group from ATP to a glucose molecule to form glucose-6-phosphate. However, like mentioned previously, glucose-6-phosphate is ionized and cannot leave or enter the cell!
Nonetheless, the first reaction (i.e., “1”) involves a nucleophilic attack of the C6-OH of glucose on the \(\gamma\) phosphate of ATP. The magnesium ion partially weakens the negative charges of the oxygen atom, making the \(\gamma\) phosphate more susceptible to nucleophilic attack and a better electrophile. The magnesium ion also forms a chelated complex with ATP.
3.2.3 Isomerization of glucose-6-phosphate to fructose-6-phosphate by phosphoglucoisomerase
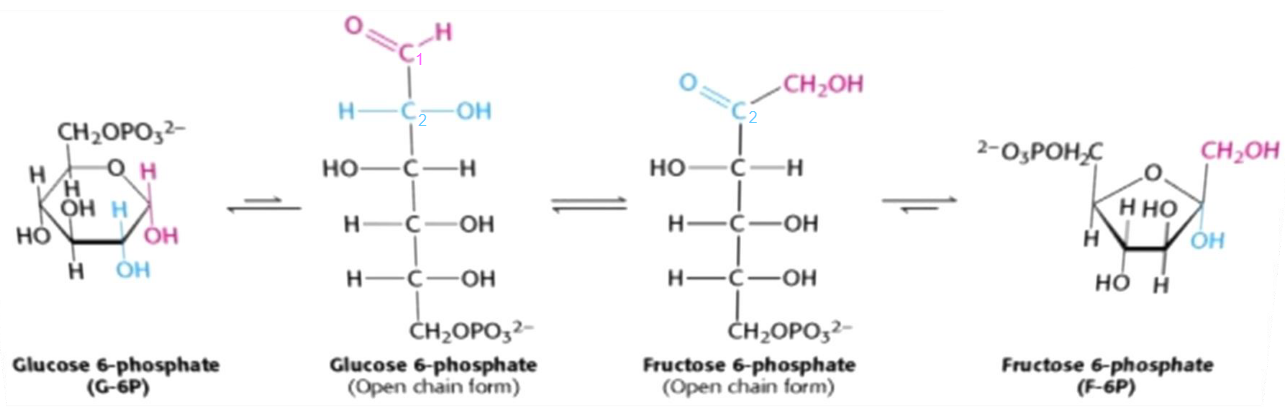
Figure 3.10: Isomerization of glucose-6-phosphate to fructose-6-phosphate
The isomerization of glucose-6-phosphate to fructose-6-phosphate is the conversion of an aldose to a ketose. This reaction is catalyzed by the enzyme phosphoglucoisomerase and has multiple steps due to the cyclic nature of both sugars.
This isomerization reaction is essential for two important reasons:
- A new, primary alcohol is formed at the first carbon (which is easily phosphorylated).
- C3 is activated, hence facilitating the carbon-carbon bond cleavage in the 4th step of glycolysis.
3.2.4 Role of phosphofructokinase-1 in glycolysis
Figure 3.11: Phosphofructokinase-1 in Glycolysis
In the third step of glycolysis, the C1-OH of fructose-6-phosphate attacks the terminal \(\gamma\) phosphate of ATP.
This reaction is generally considered to be the first committed step in glycolysis as it is thermodynamically and kinetically irreversible. Hence, phosphofructokinase-1 commits glucose to the glycolytic pathway.
Like hexokinase, phosphofructokinase-1 exists as tissue-specific lysozymes whose properties match variations in the role of glycolysis in different tissues.
3.2.5 Aldol cleavage
Figure 3.12: Aldol Cleavage
In this reaction (i.e., the fourth step of glycolysis), aldolase cleaves the fructose-1,6-biphosphate into two three-carbon compounds: dihydroxyacetone phosphate and glyceraldehyde-3-phosphate.
This reaction is an aldol cleavage.
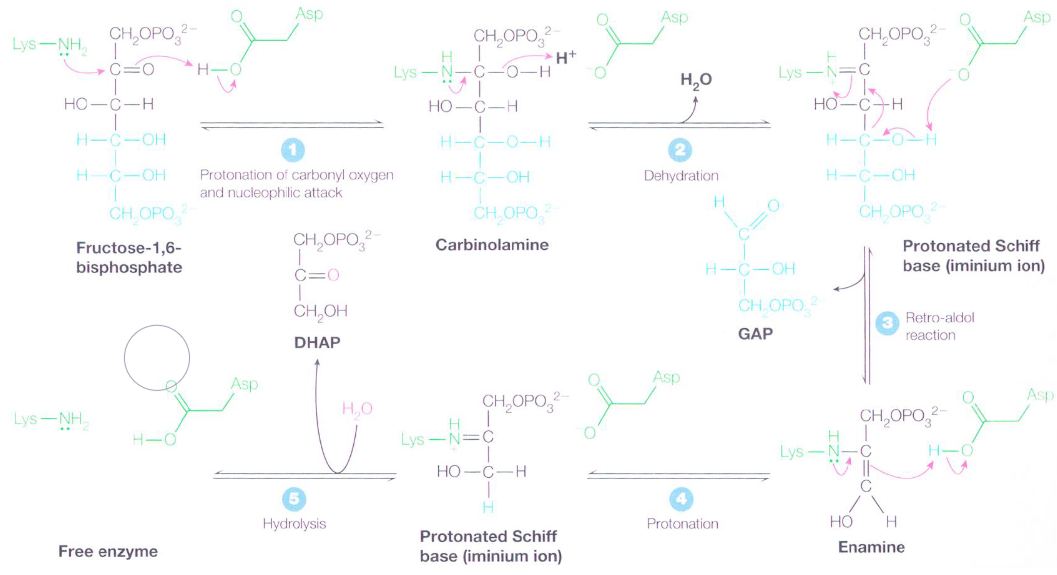
Figure 3.13: Mechanism of Aldolase
There is first a nucleophilic attack on the keto carbon with a lysine \(\epsilon\)-amino acid group in the active site - this is aided by the protonation of the carbonyl oxygen. The resulting carbinoamine then undergoes dehydration to become an iminium ion (or a dehydrated schiff base).
A reto-aldol reaction then transforms the schiff base into an enamine and glyceraldehyde-3-phosphate molecule; the enamine is then protonated to yield another iminium ion.
The schiff base’s positively-charged iminium ion is a better electron acceptor than a ketone carbonyl in facilitating reto-aldol reactions. Had glucose-6-phosphate not been isomerized to fructose-6-phosphate, a two carbon and a four carbon compound would have resulted.
3.2.6 Energy payoff phase
Figure 3.14: Energy Payoff Phase of Glycolysis
In this phase, glyceraldehyde-3-phosphate dehydrogenase first catalyzes the transformation of glyceraldehyde-3-phosphate into 1,3-biphosphoglycerate by oxidizing the carboxyl group of glyceraldehyde-3-phosphate and transferring electrons to NAD+. This is followed by substrate-level phosphorylation.
In step 7 of the above graphic, ADP is phosphorylated to ATP, yielding glyceraldehyde-3-phosphate in the process.
3.2.7 Reactions of glyceraldehyde-3-phosphate dehydrogenase
Figure 3.15: Reactions of glyceraldehyde-3-phosphate
This enzyme - as mentioned in the section above - oxidizes the carboxyl group (i.e., the aldehyde) and transferring electrons to NAD+.
However, the reaction catalyzed by this enzyme is dependent on a cysteine residue at the active site of the enzyme. The high-energy phosphate from 1,3-biphosphoglycerate release the product from the cysteine residue on the enzyme and is the start of substrate-level phosphorylation.
3.2.8 Steps 8 - 10 of glycolysis
Figure 3.16: Steps 8 - 10 of Glycolysis
To transfer the low energy phosphate on 3-phosphoglycerate to ATP, it must first be converted into a high energy bond. This is done by moving the phosphate onto the second carbon before removing water to form phosphoenolpyruvate.
The final reaction converts phosphoenolpyruvate into pyruvate.
3.3 Regulation of Glycolysis
The enzymes Hexokinase and phosphofructokinase-1 are the major regulatory enzymes of skeletal muscle. The activity of pyruvate dehydrogenase in mitochondria determines whether or not pyruvate is converted into lactate or acetyl coenzyme A (i.e., acetyl CoA).
3.3.1 Regulation of phosphofructokinase-1
Phosphofructokinase-1 has a total of six binding sites: two for MgATP and fructose-6-phosphate and four for allosteric regulatory sites. The allosteric sites for phosphofructokinase-1 have an inhibitory site for MgATP and citrate and an activation site for AMP and fructose-2,6-biphosphate.
ATP binds to two different sites on phosphofructokinase-1: a substrate binding site and an allosteric site.
Figure 3.17: Reaction Velocity of Fructose-6-Phosphate Under Low and High ATP
Under physiological conditions, the concentration of ATP is usually high enough to saturate the substrate-binding site and inhibit the enzyme by binding to the ATP allosteric site.
For most phosphofructokinase-1 isoenzymes, the binding of AMP increases the affinity of the enzyme for fructose-6-phosphate.
3.3.2 Hormonal regulation for glycolysis
The regulation of glycolysis by allosteric activation or inhibition via phosphorylating enzymes is generally a short term activity (i.e., over minutes or hours).
Consuming meals that are rich in carbohydrates causes an increase in the amount of phosphofructokinase, glucokinase, and the amount of pyruvate kinase in the liver: these changes reflect a change in the levels of gene transcription and protein synthesis.
The inverse of the aforementioned statements can also be said for periods of fasting or when levels of plasma glucagon are high!
3.3.3 What else can glycolysis do?
Glycolysis is also a precursor for many biosynthetic pathways. Intermediates can be converted into ribose-5-phosphate, UDP-glucose, mannose, and sialic acid.
Figure 3.18: Intermediates of Other Pathways from Glycolysis
Serine is synthesized from 3-phosphoglycerate and alanine from pyruvate. Glycerol-3-phosphate (the backbone of triacylglycerols) is derived from dihydroxyacetonephosphate.
3.4 Anaerobic Glycolysis
Anaerobic glycolysis happens when oxygen supplies in the cell are limited.
Here, lactate dehydrogenase oxidizes NADH generated from glycolysis by reducing pyruvate into lactate.
The energy yield per mole of glucose from anaerobic glycolysis is much lower (i.e., 2 mols of ATP per glucose molecule) than the yield of ATP from aerobic oxidation.
Lactate is released into the blood in this pathway - it is important to note that an excess of lactate can result in lactic acidemia.
3.4.1 Oxidative fates of pyruvate and NAD+
NADH from glycolysis must be continuously re-oxidized to NAD+ to provide an electron acceptor for the glyceraldehyde-3-phosphate dehydrogenase reaction and prevent product inhibition. There are two routes for oxidizing cytosolic NADH:
- The aerobic route: shuttles transfer electrons from cytosolic NADH to the electron transport chain (a mechanism covered in later sections of this website).
- The anaerobic route: NADH is reoxidized by lactate dehydrogenase to reduce pyruvate into lactate.
The anaerobic route is cataalyzed by lactate dehydrogenase; the L-steroisomer of lactate is formed at a neutral pH:
Figure 3.19: Lactate Dehydrogenase Reaction
3.4.2 Fermented milk products
3.4.2.1 Yoghurt and dahl
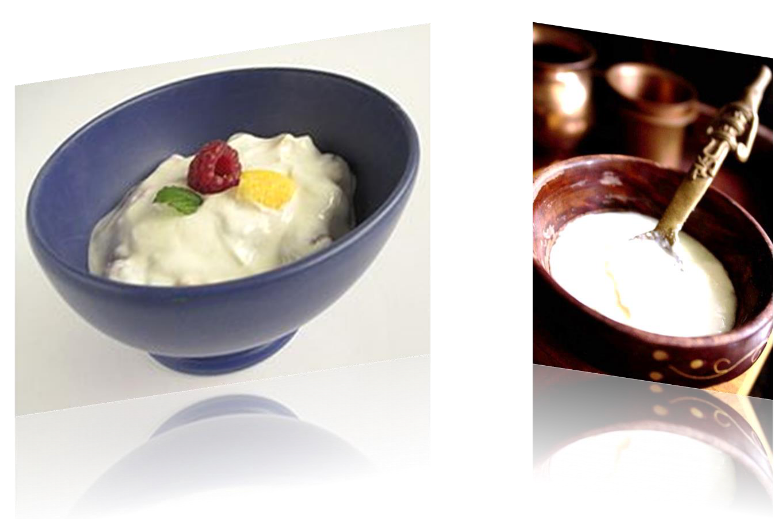
Figure 3.20: Yoghurt and Dahl
Yogurt and dahi are fermented milk products. The bacteria genuses Lactobacillus and Streptococcus are known as yoghurt cultures.
These bacteria produces lactic acid, hence giving yogurt and dahi their textures and signature tang.
3.4.2.2 Swiss cheese
The microbes Streptococcus salivarius, Lactobacillus, and Propionibacterium. The latter kind of bacteria consume lactic acid and release acetate, propionic acid, and CO2. The CO2 then forms the bubbles in the cheese; acetate and propionic acid give the cheese its nutty and sweet flavor.
Figure 3.21: Swiss Cheese
As a general rule of thumb, the larger the hole, the pronounced its flavor (due to a longer fermentation time).
3.4.3 The Cori cycle and ATP generation in the eye
Lactate that is released from other cells are uptaken by other tissues and oxidized back into pyruvate. Pyruvate is used in the liver to synthesize glucose (which then enters the blood stream). The cycling of lactate and glucose between the peripheral tissues and the liver is called the Cori cycle.
Figure 3.22: Cori Cycle
The heart is able to use lactate as its main source of energy. During periods of exercise, lactate in the bloodstream may be used by resting skeletal muscles in the arm. In the brain, glial cells and astrocytes also produce lactate (that are used by neurons or released into the blood).
The corneal epithelium generates its ATP aerobically via mitochondria, but metabolizes them anaerobically.
Figure 3.23: Structure of the eye
Mitochondria are mostly absent from the lens of the eye; however, the lens can release lactate into the vitreous body and the aqueous humor. It has no need for oxygen and capillaries.
3.4.4 Converting pyruvate to ethanol
First, pyruvate is irreversibly decarboxylated into acetaldehyde by pyruvate decarboxylase. The enzyme also requires Mg2+ and has a tightly-bound TPP coenzyme.
Figure 3.24: Alcohol Dehydrogenase Mechanism
Alcohol dehydrogenase catalyzes the reduction of acetaldehyde into ethanol - this reaction involves the oxidation of NADH.