Chapter 6 Photosynthesis
Most of Earth’s free energy needed to drive all biological reactions on Earth are trapped from solar energy via photosynthesis.
It is estimated that 1011 tons of carbon are assimilated into organic matter by photosynthesis annually. The amount of free energy stored for this is 1.5 x 1022 kJ
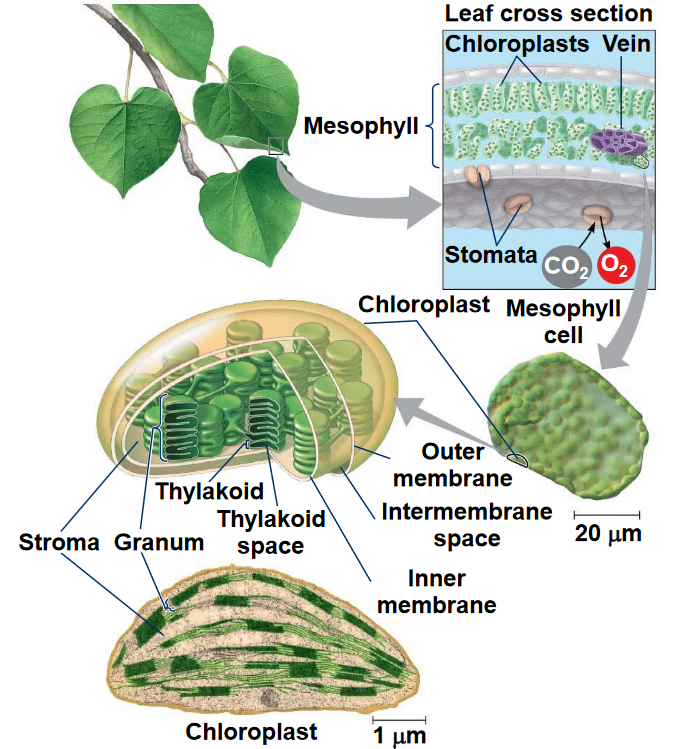
Figure 6.1: Location of Photosynthesis
6.1 Overview of Photosynthesis
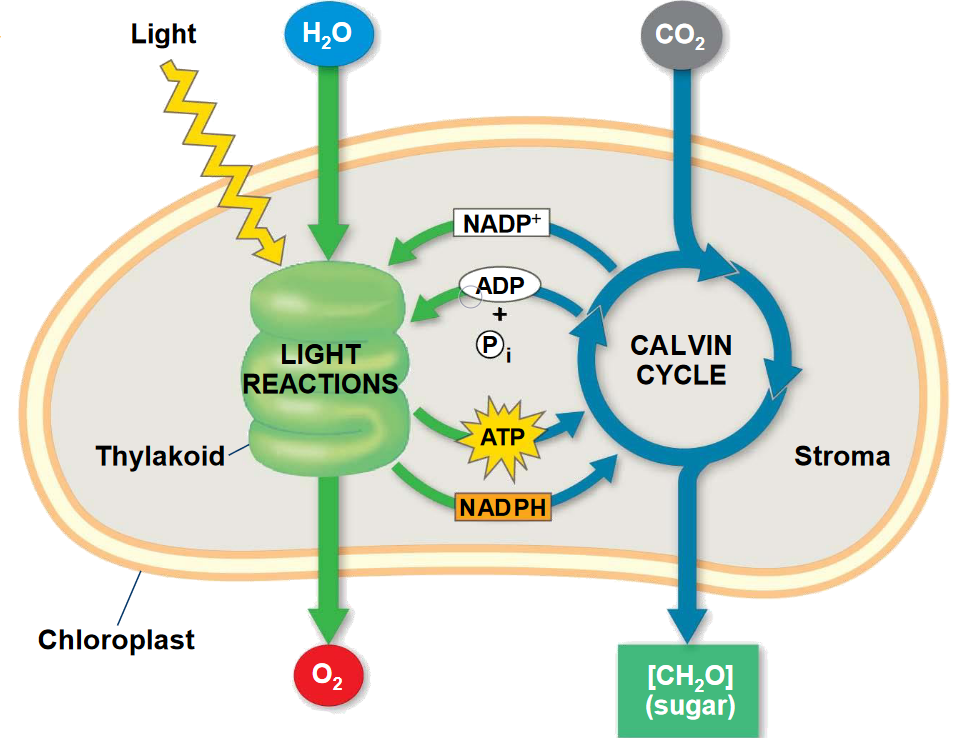
Figure 6.2: Overview of Photosynthesis
Photosynthesis has a light and a dark reaction. In the dark portion of photosynthesis and in the presence of CO2, hexose synthesis can be observed. In the absence of CO2, oxygen is evolved.
Hence, oxygen evolution can be separated from CO2 fixation and has a light dependency that CO2 fixation lacks. The light reactions of photosynthesis happens in the thylakoid membranes; the dark reactions (i.e., CO2 fixation) happens in the stroma.
6.1.1 Light and dark reactions
The overall process of photosynthesis is:
\[\begin{equation*} CO_2 + H_2O \to [CH_2O] + O_2 \end{equation*}\]
The light reaction is:
\[\begin{equation*} H_2O + NADP^+ + ADP + P_i + n(hv) \to O_2 + ATP + NADPH \end{equation*}\]
The dark reaction is:
\[\begin{equation*} CO_2 + NADPH + ATP \to [CH_2O] + NADP^+ + ADP + P_i \end{equation*}\]
The light reactions of photosynthesis generate energy rich NADPH and ATP at the expense of solar energy. These products are used in carbon fixation reactions to reduce CO2 to form trioses and more complex compounds.
6.1.2 Basic chemical reactions
Photosynthesis can be considered an oxidation-reduction reaction. In photosynthesis, CO2 is reduced and H2O is oxidized; O2 is evolved.
Note that in anoxygenic photosynthesis, H2O is not the reductant. For instance, in green-sulfur bacteria, the reactions is as follows:
\[\begin{equation*} CO_2 + H_2S \to [CH_2O] + S \end{equation*}\]
6.1.3 Energetics of photosynthesis
The standard \(\Delta G\) of glucose oxidation to CO2 is -2870 kJ / mol:
\[\begin{equation*} C_6H_{12}O_6 \to 6CO_2 + 6H_2O \end{equation*}\]
Hence, the standard \(\Delta G\) required to reduce one mole of CO2 is 480 kJ
In photosynthesis, the source of this energy is light. Hence, the basic requirement is:
\[\begin{equation*} CO_2 + 2H_2O + n\text{ photons} \to [CH_2O] + H_2O + O_2 \end{equation*}\]
Light is required as H2O is a poor electron donor (\(E^\omicron = 0.815V\)).
6.1.4 Photosynthetic pigments
Chlorophyll is an excellent absorber of light because of its aromaticity:
Figure 6.3: Structure of Chlorophyll and its Absorbance
The pigment has delocalized \(\pi\) electrons above and below its structure. When light energy is absorbed, an electron is promoted to a higher orbital, hence enhancing the potential for transfer of this electron to a suitable accpetor.
As seen in the above graphic, the absorption spectra of chlorophyll a and chlorophyll b are somewhat different.
6.1.4.1 Other pigments
Accessory light harvesting pigments increase the absorption of light of the proper wavelength.
Carotenoids and luteins posess multiple conjugated double bonds and are able to absorb visible light:
Figure 6.4: Structure of Lutein and beta-Carotene
Carotenoids have two primary roles: light harvesting and the destruction of reactive oexygen species that arise as a byproduct of photosynthesis.
6.2 Photosystems
Light absorption happens in the stroma in photosystems:
Figure 6.5: Light Harvesting Complexes
6.2.1 Light absorption and energy transfer
Figure 6.6: Molecule Excitation
Molecule I in the above graphic is excited to a higher energy state by absorbing a proton.
Furthermore, if there is a suitable molecule close to it, the energy carried by the proton can be transferred over to the “suitable molecule.” When this occurs, molecule I goes back to the ground state and molecule II goes to the excited state. This process occurs among the light-harvesting pigments (e.g., chlorophyll, carotenoids, luteins, etc).
The above is called photochemistry. In the above graphic, molecule I becomes postiively charged and molecule II becomes negatively charged. The molecule that performs photochemistry is called the reaction center molecule.
6.2.1.1 Light harvesting complexes
Figure 6.7: A Photosynthetic Unit
A photosynthetic unit can be thought of as an antenna of several hundred light-harvesting chlorophyll molecules with a pair of photochemically reactive chlorophyll a molecules - this is known as the reaction center. The purpose of the vast majority of chlorophyll in a photosynthetic unit is to harvest light and funnel it (via resonance energy transfer) to the chlorophyll pairs in the reaction center.
The oxidation of chlorophyll also leaves a cationic free radical ChI+ whose properties as an electron acceptor have important consequences for photosynthesis.
6.2.2 Light reactions and chemiosmosis
Figure 6.8: PSI and PSII in the Granum
There are two photosytems for generating O2 from water: photosystems I and II.
Photosystem I (i.e., PSI) uses ferredoxin as terminal electron acceptors (whereas photosystem II [i.e., PSII] uses quinones for such purposes).
PSIs are defined by their reaction center chlorophylls - they have a maximum red light absorption at around 700 nm. PSIIs have maximum red light absorption at around 680 nm. For this reason, the reaction center of PSIs are referred to as P700s; the reaction center of PSIIs are called P680s for the same reason.
PSIs provide NADPH (i.e., reducing power) whereas PSII splits water to evolve O2 gas and transports electrons into an electron transport chain that couples PSII and PSI.
6.2.2.1 Photosynthetic transfer
The redox components of PSII and PSI are arranged in terms of their standard reduction potentials - the resulting arrangement forms a zig-zag pattern called the Z scheme:
Figure 6.9: Z-Scheme
The Z scheme shows the pathway of electron transfer from H2O (the lower left) to NADPH (the far right) in noncyclic photosynthesis. The standard reduction potentials of each electron carrier is reflected on the position of the vertical arrows.
One photon is needed per electron in both PSI and PSII. The electrons then flow downhill through the carrier chains. Protons also move across the thylakoid membranes during the water-splitting reaction and during electron transfer via the cytochrome b6f complex - this produces a proton gradient that it key to forming ATP.
Figure 6.10: Analogy for Linear Electron Flow
In case one needs additional help understanding the previous paragraphs, provided above is an analogy for the linear flow of electrons during the light reaction.
6.2.2.1.1 Structure and function of plastoquinone in PSII
All electrons flow from pheophytin to plastoquinone to a pool of plastoquinone in the membrane. Plastoquinone is mobile in the membranes and shuttles electrons from the PSII to the cytochrome b6f complex.
Note that plastoquinone is an analog of coenzyme Q (i.e., the lipophilic electron carrier that carries electrons from complex I and IIs and shuttles them to complex III in the electron transport chain).
6.2.2.1.2 What is the cytochrome b6f complex?
Figure 6.11: The Cytochrome b6f complex. All heme groups are shown in red; all iron-sulfur clusters are shown in blue.
The cytochrome b6f complex has 26 transmembrane \(\alpha\)-helices and is structurall similar to the cytochrome bc1 complex (i..e, complex III) of the mitochondria.
It has two heme-containing electron transfer proteins (and iron-sulfur clusters that can also participate in electron transport). The whole purpose of this complex is to mediate the transfer of PSII to PSI and pump H+ across the thylakoid membrane via the Q-cycle.
6.2.2.2 Plastocyanin
Figure 6.12: Plastocyanin in the Z-Scheme
Plastocyanin (i.e., PC) is a 10.4 kDa protein that is bound to a Cu atom. PC function as a single electron carrier and undergoes alternate oxidations between Cu+ and Cu2+. When P700 is oxidized by transferring its electrons to an adjacent chlorophyll a molecule, P700+ is formed.
P700+ also readily gains an electron from plastocyanin.
6.2.3 Energetics of the two light reactions
In summary, the following equation can be used to sum up the transfer of protons in PSI and PSII:
\[\begin{equation} 2Fd(red) + H^+ + NADP^+ \rightarrow 2Fd(ox) + NADPH \end{equation}\]
6.3 Photophosphorylation
Figure 6.13: Photophosphorylation
6.3.1 Chemiosmosis in photophosphorylation
Figure 6.14: Photophosphorylation Experiment Setup
In an experiment conducted by Andre Jagendorf and Ernest Uribe in 1966, both scientists reasoned that if photophosphorylation were driven by an electrochemical gradient, that the gradient could be generated via incubating chloroplasts in an acid bath in the dark before quickly raising the pH of the external medium.
This quick change in pH should mimic conditions found in the chloroplasts and provide the means necessary for ATP generation.
To test this hypothesis, both scientists placed isolated mitochondria in a weakly acidic medium (pH 4) for a minute before the pH was raised to 8. When ADP and Pi were added, ATP generation was then observed as the pH gradient fell.
The collapse of the gradient is the energy transducing medium - the chemical potential of a concentration difference is transduced into the synthesis of ATP.
6.3.2 Cyclic photophosphorylation
Figure 6.15: Cyclic Photophosphorylation
In cyclic photophosphorylation, the “electron hole” generated in P700+ is filled by a cyclic pathway where the photoexited electron ultimately returns to P700+.
This pathway diverts activated electrons lost from PSI back to the PQ pool, the cytochrome b6f complex, and plastocyanin to re-reduce P700+.
Here, ATP is the sole product of energy conversion. Because PSII is not invovled, NADH is not generated - no O2 gas is also evolved.
6.4 Calvin Cycle
CO2 fixation is done by adding CO2 molecules one at a time to the molecule ribulose-1,5-biphosphate (i.e., RuBP) via the Calvin cycle.
Figure 6.16: Calvin Cycle
The above cycle results in the formation of hexoses and the regeneration of RuBP. The cycle also has two parts:
- Stage I traps CO2 as a carboxylate and reduces it to the aldehyde-ketone level found in sugars (hence resulting in net carbohydrate synthesis).
- Stage II regenerates RuBP.
6.4.1 CO2 fixation in the Calvin cycle
Figure 6.17: Enediol Intermediate in CO2 Fixation
The true substrate for CO2 fixation is the enediol intermediate. The product is then hydrated to yield two molecules of 3-phosphoglycerate - this reaction is essentially irreversible:
Figure 6.18: Rubisco Structure
Ribulose biphosphate carboxylase (i.e., rubisco) catalyzes CO2 fixation. Clusters of small subunits (i.e., orange and red) are located at each end of the octamer. Actives sites are labelled in yellow.
6.4.2 Stage 1 of the Calvin cycle
In the first stage, a molecule of CO2 has already been fixed to a three-carbon monosaccharide. For each CO2 molecule that passes through the reactions in the first stage of the Calvin cycle, two molcules of ATP are hydrolyzed and two molecules of NADPH have been oxidized.
6.4.3 Stoichiometry of the Calvin cycle
Figure 6.19: Stoichiometry of the Calvin Cycle
In six turns of the Calvin cycle, six RuBP molecules would have yielded 12 molecules of G3P (i.e., glyceraldehyde-3-phosphate).
Of the six glyceraldehyde-3-phosphate molecules, three molecules of fructose-1,6-phosphate are produced (of which one makes the next hexose product in the next six turns). Two molecules of fructose-6-phosphate are then used to regenerate ribulose-5-phosphate (i.e., Ru5P) which are then phosphorylated to regenerate the required six molecules of RuBP.